Abstract
In humans, movement requires a well-coordinated control of sensory and motor mechanisms, yet mundane tasks such as walking or sitting upright could drastically be affected by neurological pathologies. Despite the common belief, there is no specific centre in the nervous system dedicated to gait and postural control alone, but clusters of neurons from multiple domains and their connectivity profiles that determine adequate motor output for optimal motor performance. This article attempts to summarize the available observational and experimental evidence, and identify the neural correlates of the motor system, in order to asses the cortical and subcortical changes in stroke and movement disorders and the subsequent motor behaviour, and at the attend we attempt to answer the following question: Do motor and Cognitive Processes differ from one another?
Table of Contents 1- Neuroanatomy of motor system 2- Sensorimotor Subcortical Coordination in voluntary and involuntary movements 2a. Brainstem and spinal cord 2b. Feedback vs Feedforward motor controlIs walking voluntary or involuntary? 2c. Is walking voluntary or involuntary? 3- Do motor and cognitive Processes differ from one another? 3a. Motor behaviour and cognitive reserve 3b. Motor planning 4- Pathophysiological changes in chronic movement disorders and stroke 5- Discussion and Conclusion 6- References |
1- Neuroanatomy of motor system
In order to asses the role of motor system, we first need to distinguish between the systems and networks that underlie movement based on two inherently different domains; voluntary and involuntary movements. In voluntary control of movements, the human brain utilizes the conscious motor networks. These movements are costly and occupy a portion of our attentional resources, and predominantly involve higher cortical regions involved in error detection/correction (i.e., threading a needle). The involuntary control of movements on the other hand differs in a sense that rhythmic movements such as breathing are mainly controlled by autonomous processes in the brainstem and spinal cord. These involuntary motor outputs are also called reflexive, because by definition, reflexes are stereotyped responses to specific stimuli that are generated by simple neural circuits in the brain stem or spinal cord. Despite such distinct mechanisms between voluntary and involuntary control of movements, it is important to note, that many motor outputs can be controlled via both systems. Imagine deep breathing while meditation, we take control of an involuntary process of breathing, using the same muscle groups but different networks and pathways involved in conscious or voluntary motor control.
Taking a step back from different types of motor control, neural correlates of motor commands almost always require an integration between sensory and motor information. As a matter of fact one can think of the sensory system as the basic element of motor control, in which sensory information prime the motor system for the appropriate output. External (extrinsic) information about the environment and the internal (intrinsic) information regarding the body brought in via the sensory system (via thalamus) compared with experience-dependent information already stored in higher cortical regions involved in motor planning, such as the supplementary motor area (SMA), posterior parietal cortex (PPC), the primary motor cortex and other subcortical regions involved in procedural memory such as the basal ganglia and the cerebellum. As shown in Figure 1, It is only after such intricate mechanisms that a motor output is generated. Note that involuntary movements mainly generated in the brainstem and spinal cord are hierarchically lower than the main voluntary movement centres in higher cortical regions, hence their lower resource demand.
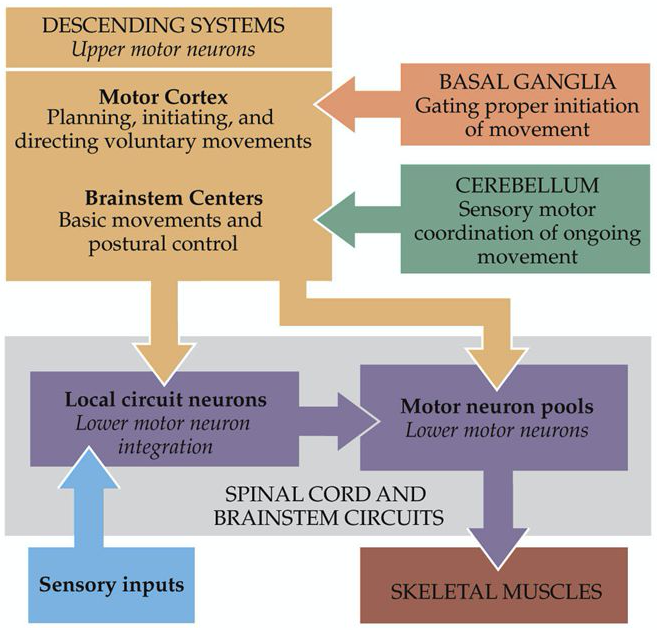
It is worth mentioning that the general consensus amongst researchers is that higher cortical motor regions such as the motor cortex consisting of the primary motor cortex (M1), premotor cortex and the supplementary motor area, are packed with pyramidal cells; one of the largest neuron types in the central nervous system. These pyramidal cells are thought to run some of their axons directly to the spinal cord and are involved in generating signals to the effector organs (i.e., leg muscles), and are important for motor planning, force control and many other motor related functions. These regions constitute only a small part of the procedural memory system, where motor skills are learned, practised and perfected through statistical trial and error learning.
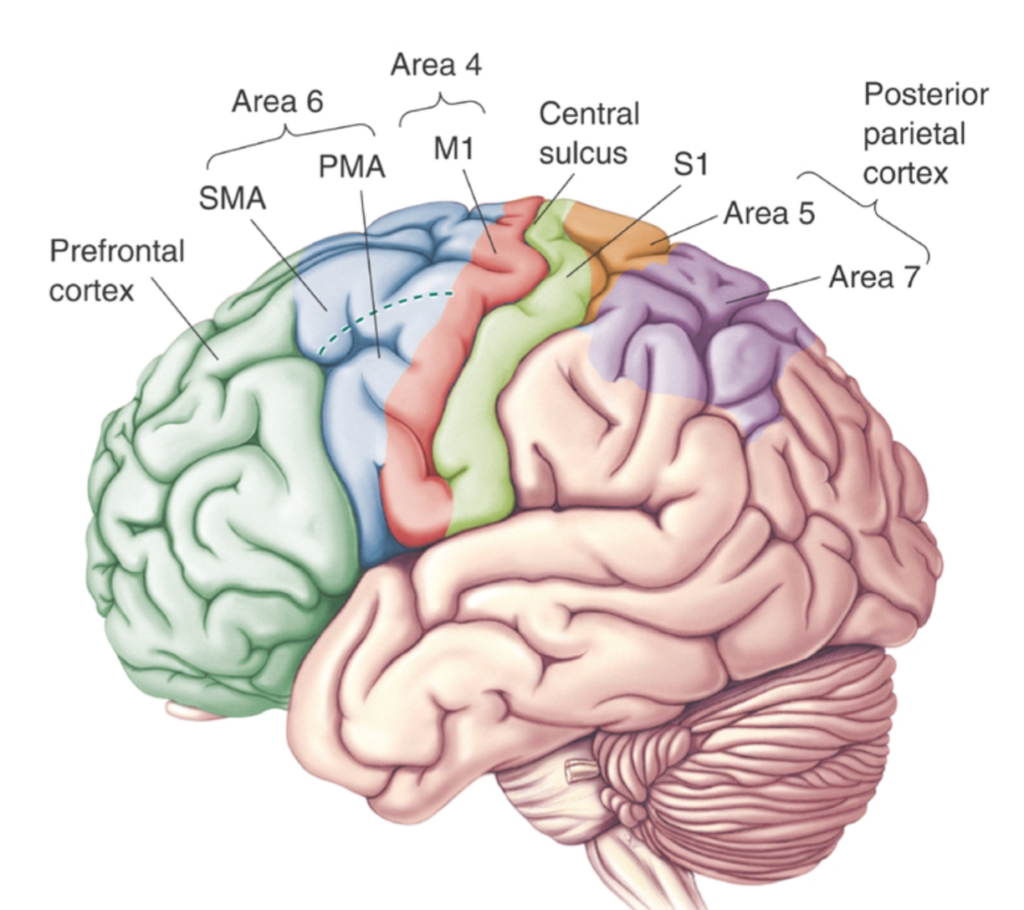
2- Sensorimotor Subcortical Coordination in voluntary and involuntary movements
As mentioned above, voluntary movements are controlled directly via the connections between the cortex and the spinal cord. As shown in Figure 3, the motor information carried in the corticospinal tract is significantly modulated by both sensory information and information from other motor regions. A continuous stream of tactile, visual, and proprioceptive information is needed to make voluntary movement both accurate and properly sequenced. In addition, the output of the motor cortex is under the substantial influence of other motor regions of the brain, including the cerebellum and basal ganglia, structures that are essential for smoothly executed movements. These two subcortical centres provide feedback essential for the smooth execution of skilled movements and thus are also important for motor learning, and are involved in procedural memory formation.
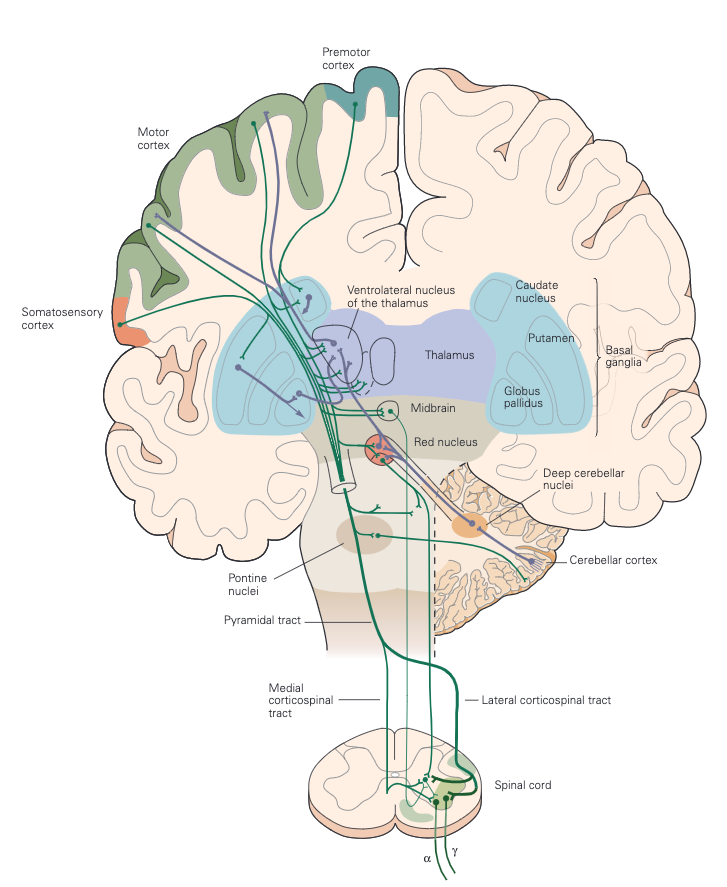
The basal ganglia are a collection of subcortical nuclei that receive direct projections from much of the neocortex, including sensory, motor, and premotor areas, and those parts of association cortex that are important for motivation, cognition, and emotion (limbic). The output nuclei of the basal ganglia send signals to regions of the thalamus that project to the cerebral cortex. Although the functions of the basal ganglia have remained surprisingly elusive, dysfunction of the basal ganglia results in particularly striking disorders of movement characteristic of Parkinson disease (tremor at rest, rigidity, and disinclination to move) and of Huntington disease (choreiform movements).Thus an important consequence of basal ganglia dysfunction is that the abnormal signals sent to cortical motor areas have a major, negative, impact on motor output. Indeed, cortical lesions that limit voluntary movement also abolish the involuntary movements associated with disorders of the basal ganglia.
Moving farther dawn, the cerebellum can also influence posture and movement through its connections in the brain stem’s motor nuclei, which can directly modulate spinal motor circuits. However, the major influence of the cerebellum on movement is through its connections in the ventrolateral nuclei of the thalamus, which connect directly to the motor and premotor cortex (Figure 3)
2a. Brainstem and spinal cord
Major player in both voluntary and involuntary movements, the medial brain stem system originates in portions of the reticular formation, vestibular nuclei, and superior colliculus. This system receives information from the cortex and other motor centres for the control of posture and locomotion. The lateral brain stem system originates from the red nucleus. It receives input from the cortex as well but is involved in the control of arm and hand movements. Spinal motor circuits are not regulated solely by descending commands. Reflex circuits and pattern generators within the spinal cord can coordinate stereotyped movements such as stepping without descending signals (Figure 4). A newborn infant, whose descending pathways cannot yet control the spinal cord, is able to execute stepping movements when lifted into the air. Descending systems coordinate reflex and patterned movements generated by spinal motor circuits and can even create new patterns of muscle activation through direct action on motor neurons. This cortical control enables greater flexibility of movements than is possible through exclusively local coordination among the spinal motor circuits. As mentioned earlier, the cortical motor areas and brain stem in turn receive input from two major subcortical structures: the cerebellum and basal ganglia. These two structures provide feedback essential for the smooth execution of skilled movements and thus are important for motor learning, the improvement in motor skills through practice. The cerebellum and the basal ganglia store memory for unconscious motor skills through pathways that are separate from those used to store factual memories of events that can be recalled consciously. The cerebellum receives somatosensory information directly from primary afferent fibres arising in the spinal cord as well as information about movement from corticospinal axons descending from the neocortex. The basal ganglia receive direct projections from much of the neocortex, which supply both sensory information and information about movement as one of the major coordination centres in the brain.
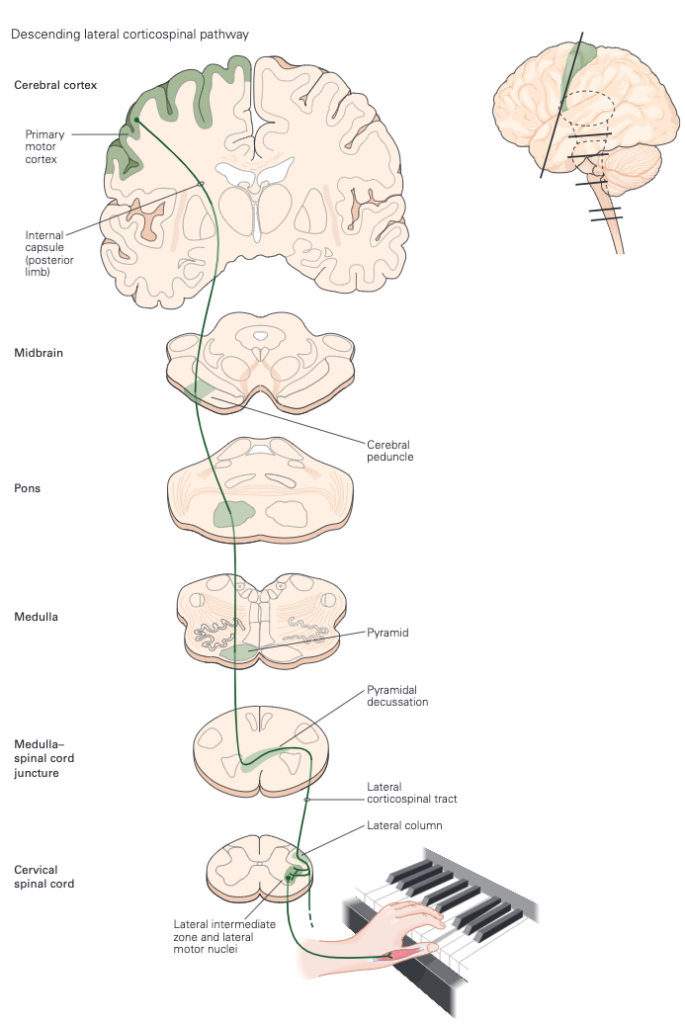
2b. Feedback vs Feedforward motor control
Motor behaviours can occur along a continuum of control, ranging from feedback to feedforward. Feedforward movements are made without the online use of sensory feedback evolving during the action, and require an internal model for accuracy (Kawato, 1999). Such actions can occur rapidly, as there is no need to account for the delay of feedback loops. Feedback control, in contrast, involves modification of the ongoing movement using information from sensory receptors. This type of control allows for a high degree of accuracy, as well as for error detection and correction, but is necessarily slow. Optimal movement control likely reflects a combination of both feedback and feedforward processes (Desmurget & Grafton, 2000).
Many studies have investigated the contributions of cortical and subcortical regions to motor control by varying factors such as movement rate (Deiber et al., 1999), force , or motor error (Kitazawa et al., 1998), and observing the neural responses. By independent manipulation of these variables, changes in force output, for example, could be dissociated from other performance effects such as velocity or motor error. Such experiments have contri- buted greatly to our understanding of the neural coding of movement variables. Under natural conditions, however, these motor variables interact in a complex manner to produce states of behavior, such as when subjects shift along the continuum of feedback and feedforward control.
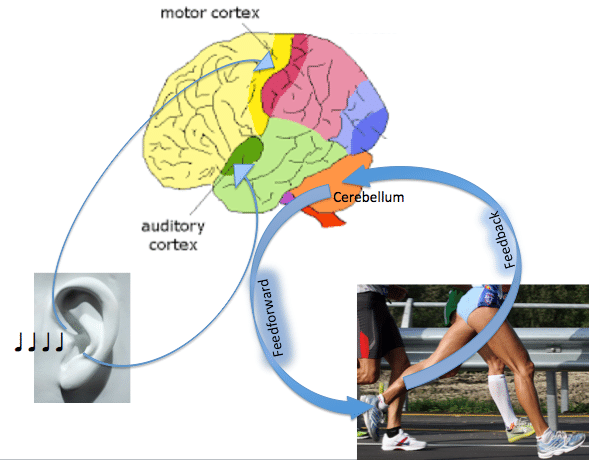
A PET study by Winstein et al. (1997) examined the neural substrates associated with degree of motor planning by having subjects perform the classical repetitive aiming task of Fitts (1954). Fitts’ Law describes the well-known speed–accuracy tradeoff; that is, increasing the amplitude of a movement or decreasing the target size increases the index of difficulty, resulting in prolonged movement duration. Winstein et al. (1997) found that increasing task difficulty was associated with greater activation in bilateral dorsal premotor cortex, SMA, caudate nucleus, and visual and parietal cortical areas. In contrast, decreasing task difficulty was associated with increasing activation in ventral premotor cortex, the anterior cerebellum, and temporal and visual cortical regions. Thus, parametric modulation of task difficulty, and therefore degree of motor planning, resulted in dissociable networks of activation. The task utilized in this study was continuous aiming with a hand-held stylus. Since evidence indicates that continuous and discrete actions may not be controlled in the same manner (Diedrichsen et al., 2002; Kennerley et al., 2002), it remains unclear whether performance of discrete actions would yield the same pattern of results under the Fitts’ manipulations.
One defining feature of feedback control is the capacity to correct for motor errors during the course of an ongoing movement. Desmurget et al. (1999) have determined the under- lying neural circuitry contributing to movement corrections by introducing a step-change in target location after a saccade has been initiated (Goodale et al., 1986). In this case, the correction is considered nonvisual since subjects do not have visual feedback of the moving limb. This work, utilizing PET and transcranial magnetic stimulation (TMS), demonstrates that the posterior parietal cortex plays a critical role in these non-visual feedback loops (Desmurget et al., 1999). In addition to non-visual feedback control, many actions in daily life require visually based corrections, when one can see both the target and the moving limb. It is unknown whether the same posterior parietal region contributes to this type of corrective action as well.
Movement control changes from feedback to feedforward during the course of skill acquisition. Movements become faster and more accurate as a function of practice, and the learner becomes less reliant on feedback control (Pratt et al., 1994). During skill acquisition, this shift of control strategy is accompanied by the formation of a representation of the newly learned movement. When task difficulty is changed in a counterbalanced fashion, such as in the Winstein et al. (1997) study, these differing modes of control can be observed in the absence of skill learning.
2c. Is walking voluntary or involuntary?
In order to answer this question, we need to rely on the information provided above and the neural correlates involved in both voluntary and involuntary movements. If we consider walking as an automated rhythmic and alternating act of moving one’s limbs, it might not be too far off to call it an involuntary movement. However, walking as an output of the motor system differs greatly with the mere interpretation of observed repetitive act of moving lower and upper extremities. Despite the fact, that many processes involved in the task of walking require a control of posture and balance via the brain stem and the spinal cord (automatic), as we move and interact with our environment the simple act of walking that at first might seemed reflexive, becomes highly influenced by higher cortical and subcortical regions. Given this complex relationship between multiple regions in the central nervous system, assigning voluntary or involuntary status to walking can only be done if we break it down in multiple different phases. Imagine you are walking home from the supermarket, carrying a grocery bag, and talking on your phone. As you walk around while still carrying a bag in your left hand and the phone in your right hand, you can still maneuver around obstacles such as other pedestrians, trees, lamp posts, curbs and so on, yet pay attention (some people don’t) to traffic lights, recognize faces, navigate your way, perceive and estimate speed of moving objects (estimate when you can jaywalk as cars approaching), but walking still continues. Now, going back to the definition of voluntary and involuntary movements, we realize that it is not so simple to assume “walking” as a voluntary or involuntary process but a mixture of both (Yuan et al., 2016). An easy way out is to think of the initiation phase as a voluntary process and the the rest involuntary until reaching an obstacle, changes in environment or the end of the walk which is yet again voluntary? The answer may lie in the role of default mode network and the conservation of attentional resources which will be discussed in the following sections.
3- Do motor and cognitive Processes differ from one another?
3a. Motor behaviour and cognitive reserve
If we consider the specialized role of each entity involved in the motor system, it may seem counterintuitive to include motor behaviour and cognitive abilities in the same category. Just as the literature in motor neuroscience draw a line between cognitive and motor processes. But here I argue, that motor competency directly influences the cognitive reserve and vice versa. Before delving deeper, let us define a few terms. Cognitive reserve is mainly refers to the capacity of an individual brain to perform cognitively optimal in the context of ageing or neuropathology. A healthy motor system requires a nervous system that is well-tuned and have access to higher cognitive processors to intervene and modify motor outputs when needed. Going back to the walking example, it is impossible to walk continuously while talking on the phone, carrying a bag in one hand and the phone in another, while in the mean time paying attention to traffic signs, and finding your way home when either one or both motor or cognitive circuits function sub-optimally . Whether motor and cognitive processes run in parallel or they use the same networks and pathways is the question that will be answered shortly, but before that I would like to use my observations in cognitive performance in patients with right hemispheric stroke (large vessel MCA occlusion) that perform a cognitive task in standing vs. sitting (unpublished work). When patients with math background are given a simple arithmetic task, their reaction time increases substantially in standing. The same patients also report, that despite their ability to walk independently (with walking aids) they have a difficult time walking in crowded places or places that play loud music. Many of my patients described to me a phenomenon that I would like to call it the “post-stroke deer in the headlight phenomenon”. In one account a patient described her experience while crossing the road. Five years prior to this incident, she had a right middle cerebral artery ischemic stroke which left her with significant hemiparesis of the left upper and lower extremities with no residual cognitive deficits but a noticeable hemiplegic gait.
” I took my cane to cross the road and check my mailbox, as I mainly do, a couple of times a week. I live on a quiet road, but suddenly I noticed a car from the corner of my eyes, although the car was probably travelling under the speed limit and it was far away from me, I was stuck, fully paralyzed and could not move. That was the last time I checked my mailbox alone”
Although this may fall under the jurisdiction of “fear of falling” but one can argue that such phenomena arise mainly due to a heavy burden of maxed-out attentional resources and lack of higher cortical control of motor outputs which in turn makes movements reflexive instead of controlled, and drains the cognitive reserve.
3b. Motor planning
If you ever walked in a park you may have noticed a worn path in the middle of a nicely- grassed area. Have you ever asked yourself why? Humans like many other animals
Perception of space, and even more complex cognitive acts, were once thought to be represented only in higher-order sensory and association areas of cerebral cortex. In a radical departure from previous thinking, we now believe that the premotor areas in the cortex may also have cognitive functions. At the highest levels of sensory-motor interaction, neurons do not simply encode the physical features of the sensory stimulus or the force or direction of movement. Rather, they encode something more abstract that includes features of both the object and the movement: They encode the relationship between the body and the object with respect to a particular goal. For example, in anticipation of drinking they may represent a configuration of the hand in relation to graspable features of a cup. Although picking up a cup appears to be a simple mechanical action, the neural machinery underlying it is surprisingly complex, requiring a number of preparatory steps in the parietal and frontal premotor and motor cortex.
Since we have established a relationship between the cortical motor and premotor areas in action planning, we also need to mention that the basal ganglia and cerebellum influence cortical motor circuits through connections in the thalamus. The motor cortex determines which muscle groups are activated and the magnitude of force to exert. Based on inputs from the motor cortex, basal ganglia, cerebellum, and other brain stem nuclei, the spinal cord initiates appropriate muscle contractions to accomplish purposeful movement. These circuitries are immensely important in cognitive aspect of movement or motor planning. Let us think about the following questions: When picking up an object how do we know how much force to exert? When riding a train, how do we adjust our posture, balance, and waking in order to avoid falling?

To answer these questions, an epistemological question should be asked first “how do we know what we know? Neurologically speaking, the answer lies within the early stages of the human development! omitting the role of prepackaged gene-related motor mechanisms, as explained earlier, we learn through trial and error and statistical learning. In the brief window of opportunity for learning motor tasks, humans learn how to hone their motor systems in order to interact with their environment. This learning opportunity seems to create and store vaults of information and constructs motor maps about one’s body and the world. these vaults will then serve as comparators in the central nervous system, where future sensory information will be compared with prior experiences and learned skills and an output is generated based on the compared information and prior experiences. However, as we know “practice makes perfect” . Any future interaction will lead to a much faster response, until a surprised sensory stimulus such as a sudden break on the train causing riders to loose their balance way before they have the opportunity to adjust their balance. This applies to the simple act of lifting an object too. Imagine if you were to lift a kettle that you expected it to be full of water, as you tense up your arm and hand muscles to lift the 2 litre kettle, you realize that the kettle comes right off the stove top much easier than you expected, it is only then that you realize that the kettle was empty. These two simple examples clearly show the importance of the prior knowledge in adjusting one’s motor behaviour, also emphasizes the importance of the collaboration between automated motor processes and the cognitive mechanisms involved in motor planning (such as the comparators). This exact intermingling between the incoming stimuli and endogenous comparators is also seen in day to day tasks such as walking and control of posture which we will be exploring in the next section.
4- Pathophysiological changes in chronic movement disorders and stroke
Whether acute or chronic, damages to any component of the motor system can create a constellation of signs and symptoms that could affect motor behaviour. Focal damages to the motor or sensory cortices due to neoplasm or stroke can directly affect localized functions to the specific region. Despite common belief, that focal damage does necessarily change other regions such as subcortical integration areas, current research suggests that many nuclei of thalamus and basal ganglia ipsilateral to the damaged areas also dysfunction, which eventually leads to atrophy in the size of the neural clusters in those regions. If we look at most neurological disorders, it is quite astonishing how many disorders manifest at least with one or more motor symptoms, emphasizing the vast allocation of the neural resources to motor processes.
Diseases of the basal ganglia on the other hand are associated with disturbances with movement, executive function, mood and behaviour. Movement disorders arise from dysfunction of the basal ganglia–thalamocortical motor circuit, ranging from hypokinetic disorders, of which Parkinson disease is the best-known example, to hyperkinetic disorders, exemplified by Huntington disease, dystonia, and hemiballism. Direct damage to the basal ganglia, more specifically to the substantia nigra can lead to hypokinetic disorders such as Parkinson’s disease. There are hundreds of other motor-related disorders such as multiple sclerosis and amyotrophic lateral sclerosis, but If we look at most neurological disorders, it is quite astonishing how many disorders manifest with at least one or more motor symptoms, emphasizing the vast allocation of the neural resources to motor processes.
5- Discussion and Conclusion
As we saw earlier, in the central nervous system, each muscle and joint is represented by a column of neurons whose axons branch and terminate in several functionally related spinal motor nuclei (this branching is minimal for cortical cells that control distal muscles because these muscles require more independent control). The cortical neurons also form synapses with interneurons in the spinal cord. These connections allow voluntary movements to switch on entire spinal circuits—the motor neurons, interneurons, and central pattern generators that execute reflex actions. These circuits are then able to integrate and convert local sensory input to motor output without further direction from cortical centres. Fine tuning and coordination of movements are now in the hands of subcortical regions such as the basal ganglia and the cerebellum, which masterfully control the sequences of signal delivery to the effector organs via spinal cord and the peripheral nervous system.
Although in neurology, localizing lesions based on clinical manifestations is a daily task, and with the advent of neuroimaging, it has become increasingly easier to locate pathological causes underlying sub-optimal motor performance, and despite its importance, we have yet to settle the age old debate; “localization vs. Holism”. This debate plays an important role in understanding of how our brain constructs an internal map of what is out in the environment, where we are in respect to points of references determined by our senses such as vision, audition, touch, proprioception and other senses. Thinking back about the walking example the only way we can perform complex tasks is the combination of all elements in both the central and the peripheral nervous system.
One way to look at it is the concept of body schema, first introduced by Henry Heads and Gordon Morgan Holmes in 1911. A body schema is the collection of processes that registers the posture of one’s body parts in space. The schema is updated during body movement. This is typically a non-conscious process, and is used primarily for spatial organization of action. It is therefore a pragmatic representation of the body’s spatial properties, which includes the length of limbs and limb segments, their arrangement, the configuration of the segments in space, and the shape of the body surface. Body schema also plays an important role in the integration and use of tools by humans (Gurfinkel et al., 1988). Now, considering this concept, it would be difficult not to disregard the role of cognition in motor behaviour. Even if cognitive processes are not necessarily involved in “involuntary movements” per se, given the finite processing power in the brain, once pathologies affect parts of these so called “involuntary motor circuits” cognitive domains are also affected. This not not unique to cortical pathologies, subcortical pathologies such as thalamic and basal ganglia strokes, severely affect multiple cognitive domains. It is therefore quite possible to draw a conclusion about the thin line between the motor and cognitive domains, perhaps there is no “line” in between. And revision to our current neurorehabilitation models is detrimental.
References:
Bansil, S., Prakash, N., Kaye, J., Wrigley, S., Manata, C., Stevens-haas, C., & Kurlan, R. (2012). Movement Disorders after Stroke in Adults: A Review. Tremor and Other Hyperkinetic Movements, 2. http://www.ncbi.nlm.nih.gov/pmc/articles/PMC3570045/
Clark, I., & Tamplin, J. (2016). How Music Can Influence the Body: Perspectives From Current Research. Voices: A World Forum for Music Therapy, 16. https://doi.org/10.15845/voices.v16i2.871
Deiber, M. P., Honda, M., Ibañez, V., Sadato, N., & Hallett, M. (1999). Mesial motor areas in self-initiated versus externally triggered movements examined with fMRI: Effect of movement type and rate. Journal of Neurophysiology, 81(6), 3065–3077. https://doi.org/10.1152/jn.1999.81.6.3065
Desmurget, M. & Grafton, S. Forward modeling allows feedback control for fast reaching movements. Trends Cogn. Sci. 4, 423-431. (n.d.). ResearchGate. http://dx.doi.org/10.1016/S1364-6613(00)01537-0
Desmurget, M., & Grafton, S. (2000). Forward modeling allows feedback control for fast reaching movements. Trends in Cognitive Sciences, 4(11), 423–431. https://doi.org/10.1016/S1364-6613(00)01537-0
Fasano, A., & Bloem, B. R. (2013). Gait disorders. Continuum (Minneapolis, Minn.), 19(5 Movement Disorders), 1344–1382. https://doi.org/10.1212/01.CON.0000436159.33447.69
Fitts, P. M. (1954). The information capacity of the human motor system in controlling the amplitude of movement. Journal of Experimental Psychology, 47(6), 381–391. https://doi.org/10.1037/h0055392
Florman, J. E., Duffau, H., & Rughani, A. I. (2013). Lower Motor Neuron Findings after Upper Motor Neuron Injury: Insights from Postoperative Supplementary Motor Area Syndrome. Frontiers in Human Neuroscience, 7. https://doi.org/10.3389/fnhum.2013.00085
Functional Anatomy of Nonvisual Feedback Loops during Reaching: A Positron Emission Tomography Study | Journal of Neuroscience. (n.d.). Retrieved May 10, 2020, from https://www.jneurosci.org/content/21/8/2919Gait Abnormalities | Stanford Medicine 25 | Stanford Medicine. (n.d.). Retrieved June 2, 2017, from https://stanfordmedicine25.stanford.edu/the25/gait.html
Gallagher, S., & Cole, J. (1995). Body Image and Body Schema in a Deafferented Subject. The Journal of Mind and Behavior, 16(4), 369–389. JSTOR.
Giladi, N., Horak, F. B., & Hausdorff, J. M. (2013). Classification of gait disturbances: Distinguishing between continuous and episodic changes. Movement Disorders : Official Journal of the Movement Disorder Society, 28(11). https://doi.org/10.1002/mds.25672
Gurfinkel, V. S., Levik, Yu. S., Popov, K. E., Smetanin, B. N., & Shlikov, V. Yu. (1988). Body Scheme in the Control of Postural Activity. In V. S. Gurfinkel, M. E. Ioffe, J. Massion, & J. P. Roll (Eds.), Stance and Motion: Facts and Concepts (pp. 185–193). Springer US. https://doi.org/10.1007/978-1-4899-0821-6_17
Guzzetti, S., Mancini, F., Caporali, A., Manfredi, L., & Daini, R. (2019). The association of cognitive reserve with motor and cognitive functions for different stages of Parkinson’s disease. Experimental Gerontology, 115, 79–87. https://doi.org/10.1016/j.exger.2018.11.020
Kandel, E. R., Schwartz, J., Jessel, T., Sieglebaum, S., & Hudspeth, A. J. (n.d.). Principles of Neural Science, Fifth Edition | AccessNeurology | McGraw-Hill Medical. Retrieved May 10, 2020, from https://neurology.mhmedical.com/book.aspx?bookID=1049
Kaski, D., & Bronstein, A. M. (2014). Treatments for Neurological Gait and Balance Disturbance: The Use of Noninvasive Electrical Brain Stimulation. Advances in Neuroscience, 2014. https://doi.org/10.1155/2014/573862
Kawato, M. (1999). Internal models for motor control and trajectory planning. Current Opinion in Neurobiology, 9(6), 718–727. https://doi.org/10.1016/s0959-4388(99)00028-8
Kitazawa, S., Kimura, T., & Yin, P. B. (1998). Cerebellar complex spikes encode both destinations and errors in arm movements. Nature, 392(6675), 494–497. https://doi.org/10.1038/33141
Labriffe, M., Annweiler, C., Amirova, L. E., Gauquelin-Koch, G., Ter Minassian, A., Leiber, L.-M., Beauchet, O., Custaud, M.-A., & Dinomais, M. (2017). Brain Activity during Mental Imagery of Gait Versus Gait-Like Plantar Stimulation: A Novel Combined Functional MRI Paradigm to Better Understand Cerebral Gait Control. Frontiers in Human Neuroscience, 11. https://doi.org/10.3389/fnhum.2017.00106
Nanhoe-Mahabier, W., Snijders, A. H., Delval, A., Weerdesteyn, V., Duysens, J., Overeem, S., & Bloem, B. R. (2011). Walking patterns in Parkinson’s disease with and without freezing of gait. Neuroscience, 182, 217–224. https://doi.org/10.1016/j.neuroscience.2011.02.061
Pélisson, D., Prablanc, C., Goodale, M. A., & Jeannerod, M. (1986). Visual control of reaching movements without vision of the limb. Experimental Brain Research, 62(2), 303–311. https://doi.org/10.1007/BF00238849
Plenz, D., & Kital, S. T. (1999). A basal ganglia pacemaker formed by the subthalamic nucleus and external globus pallidus. Nature, 400(6745), 677–682. https://doi.org/10.1038/23281
Seidler, R. D., Noll, D. C., & Thiers, G. (2004). Feedforward and feedback processes in motor control. NeuroImage, 22(4), 1775–1783. https://doi.org/10.1016/j.neuroimage.2004.05.003
Study of gait as a biomarker for cognitive decline—Institute of Neuroscience—Newcastle University. (n.d.). Retrieved June 2, 2017, from http://www.ncl.ac.uk/ion/research/neurodegenerative/ncpdproj3/
Takakusaki, K. (2017). Functional Neuroanatomy for Posture and Gait Control. Journal of Movement Disorders, 10(1), 1–17. https://doi.org/10.14802/jmd.16062
Tattersall, T. L., Stratton, P. G., Coyne, T. J., Cook, R., Silberstein, P., Silburn, P. A., Windels, F., & Sah, P. (2014). Imagined gait modulates neuronal network dynamics in the human pedunculopontine nucleus. Nature Neuroscience, 17(3), 449–454. https://doi.org/10.1038/nn.3642
Terrier, P. (2012). Step-to-Step Variability in Treadmill Walking: Influence of Rhythmic Auditory Cueing. PLOS ONE, 7(10), e47171. https://doi.org/10.1371/journal.pone.0047171The walking brain | Atlas of Science. (n.d.). Retrieved June 2, 2017, from https://atlasofscience.org/the-walking-brain/
Verghese, J., Ambrose, A. F., Lipton, R. B., & Wang, C. (2010). Neurological Gait Abnormalities And Risk Of Falls In Older Adults. Journal of Neurology, 257(3), 392–398. https://doi.org/10.1007/s00415-009-5332-y
Winstein, C., Lewthwaite, R., Blanton, S. R., Wolf, L. B., & Wishart, L. (2014). Infusing Motor Learning Research Into Neurorehabilitation Practice: A Historical Perspective With Case Exemplar From the Accelerated Skill Acquisition Program. Journal of Neurologic Physical Therapy : JNPT, 38(3), 190–200. https://doi.org/10.1097/NPT.0000000000000046
Yuan, J., Blumen, H. M., Verghese, J., & Holtzer, R. (2015). Functional Connectivity Associated With Gait Velocity During Walking and Walking-While-Talking in Aging: A Resting-State fMRI Study. Human Brain Mapping, 36(4), 1484–1493. https://doi.org/10.1002/hbm.22717